Introduction: From “Cut and Burn” to Cryosections
The nomination of a new chemical entity, or NCE, during drug development triggers a cascade of activities meant to prepare the NCE for testing in humans. Because little is known about the NCE at this point, investigators launch preclinical studies to assess the chemical entity’s safety and efficacy before human clinical trials commence.
A key aspect of preclinical research is understanding a drug’s biochemical “journey” in a living system — what organs and tissues does it enter, how is it metabolized and what effects does it have at a cellular level? DMPK (Drug Metabolism and Pharmacokinetics) studies, or ADME studies, anchor this research, with the goal of determining the viability and the ‘drugability’ of a drug candidate. ADME is an abbreviation indicating the type of data revealed by the studies:
- Absorption — How much of the drug is absorbed and circulating in the bloodstream and how quickly?
- Distribution — Where is the drug distributed within the body? What compartment, muscles and/or organs does it enter? What is the rate and extent of the distribution?
- Metabolism — How fast is the drug broken down into its metabolites? What is the mechanism of action of its metabolic fate? Are any metabolites active or toxic?
- Elimination — Is the drug excreted through the kidneys or through feces, lungs or skin? How quickly?
ADME studies are often accompanied by PK and TK analyses, which provide additional information about the behavior of a drug in a living system.
- Pharmacokinetics — How does the drug move throughout the body at the pharmacological dose?
- Toxicokinetics — How does the drug move throughout the body during toxicology evaluation at a much higher and potentially toxic dose?
Clearly, following the pathway of a chemical in a complex living system to answer these questions is a significant challenge. In the 1950s, researchers began experimenting with radiolabeling as a way to “assess” where and how a particular molecule travels through tissues, and large, complex animal systems. By “labeling” molecules with radioactive isotopes, researchers could easily track them using imaging equipment.
Today, carbon-14 (14C) and tritium (3H) labeled compounds are used to determine ADME characteristics of an NCE. However, using radioisotopes in humans poses safety and health risks. The US Food and Drug Administration (FDA) requires, minimally, lower mammalian models, such as rats, to predict radiation exposure to tissues and organs that could result from administering an experimental radiolabeled drug. This prediction process is known as dosimetry — the determination and measurement of the dosage of radiation absorbed by a substance or a living organism.
Prior to 1995, dosimetry was conducted by a process known as liquid scintillation counting (LSC). This process, also known as “cut and burn,” involved dissecting organs, homogenizing them, oxidizing or solubilizing the tissue, and then using a special analyzer to detect photons of light emitted by radioactive labels of the target drug. The analyzer then translated the photons into a count of radioactivity in the sample (and therefore a measure of the drug’s presence). This method, because it depended on accurate dissection of multiple organs and homogenized samples of the dissected organs, did not account for potential contamination nor the fact that organs are composed of different tissues. Therefore, it provided a quantitative measure of drug distribution, but it only gave one value for the whole organ.
In 1995, a new technique emerged to provide the specificity that LSC was lacking. Known as quantitative whole-body autoradiography, or QWBA, this approach begins when a radiolabeled drug is administered to a group of animal models. The animals are then euthanized at different time points and rapidly frozen. Finally, a macrotome prepares thin slices of the animals, and organ radioactivity is measured in cross section to show distribution of the drug candidate over time.
Recently, some researchers have argued that QWBA’s time has passed. They argue that imaging mass spectrometry techniques are more suitable for ADME studies. One such technique is matrix-assisted laser desorption/ionization imaging mass spectrometry (MALDI-IMS). MALDI makes it possible to directly measure a wide range of biomolecules in situ, from small metabolites to proteins, but it also presents some challenges. For example, MALDI offers limited sensitivity and requires different ionizing matrix and solvent conditions to identify and quantify the various compounds of interest, making it difficult to assure the quality of drug/metabolite quantitation.
More importantly, the FDA currently mandates the use of radiolabeled drugs for ADME studies, but it also requires that investigators demonstrate that the radioactivity from the radiolabeled drug will not harm humans. For this reason, the FDA and other regulatory authorities recommend the use of QWBA for use in dosimetry studies. This reaffirms QWBA as the gold standard for ADME, especially when it’s coupled with other techniques, such as quantitative autoradioluminography (QARL) and micro autoradiography (MARG).
This white paper will explore why QWBA is still the best preclinical imaging technique, what to look for in DMPK labs that provide the service, and how it integrates with QARL and MARG to provide a comprehensive picture of drug distribution for a particular NCE.
QWBA: Whole-Body with Digital Autoradiographic Detection
Before there was QWBA, there was just WBA — whole body autoradiography. Introduced in 1954, WBA finally gave investigators a true spatial, time-sequenced view of a drug’s metabolic pathway. WBA starts by administering a radiolabeled compound (often 14C, but also 3H; sulfur-35, 35S; and iodine-125, 125I, have been used more often with complex biological drug candidates) to a group of laboratory animals. The animals are euthanized and quick-frozen. Euthanasia at different times after dosing can determine the PK of the drug candidate (in reality, it is the total drug-related radioactivity) through tissues. Each frozen carcass is embedded in a support matrix such as carboxymethylcellulose and sectioned, at a thickness between 20 and 50 µm. The sections are then dehydrated and, finally, mounted on X-ray films and exposed for several weeks. See Figure 1.
When energy from radiolabeled molecules strikes the X-ray film, the energy is converted into light, which then creates a latent image in the photographic film. By studying the location and density of these images, it’s possible for investigators to see where the drug candidate is distributed and where it is concentrated. Obtaining true quantitative data from these X-ray images, however, is challenging. High background levels of radiation make it difficult to detect low-level radioactivity, and a saturation effect leads to poor linearity. As a result, it’s challenging to get reliable quantitative data from the autoradiography using a densitometer.
In 1975, researchers overcame these issues when they developed new imaging methods based on phosphor imaging plates (IP). The IP is a flexible, film-like sensor impregnated with aggregates of fine crystals. The crystals are photostimulable phosphors of barium fluorobromide containing traces of bivalent europium as the luminescence center, typically depicted as BaFBr:Eu2+. The radiation energy absorbed by the crystals remains stable until the plate is scanned by a laser beam, which releases the energy as luminescence.
By the early 1990s, imaging using IP combined with computer analysis came of age. First, a red laser beam is directed toward the plate by a scanning galvanometer or rotating mirror. At the same time, the beam scans across the plate so that the crystals of the storage phosphor plate are stimulated. The emitted light from the phosphors is proportional to the amount of energy stored in the plate. Finally, a photomultiplier tube receives the emitted light and transforms the light signal into an electrical signal, which is amplified, digitized and stored in a computer file.
Figure 1. WBA and QWBA share many steps: Whole-body autoradiography begins when animals receive a radiolabeled dose. The animals are anesthetized (a) and euthanized at time intervals and then flash-frozen (b). After the frozen carcasses are embedded in carboxymethylcellulose (c), they are sectioned at a thickness between 20 and 50 µm (d), dehydrated (e) and mounted on a thin cardboard support (f). X-ray film (for WBA) or phosphor imaging plate (IP for QWBA) (f) are pressed against the sections in a tight and lightproof cassette and stored in a lead safe for the length of exposure. After exposure, the X-ray film is developed, and the images are analyzed for areas of radioactivity, indicated by images on the film; IP screen processing is discussed below.
Analysis of phosphor imaging plates is known as autoradioluminography and makes it possible to quantify radioactivity in whole-body sections, transforming WBA into a truly and reliably quantitative technique for the first time. In fact, analysis of the digital images prepared using QWBA reveals concentrations of radioactivity in various tissues (see Figure 2). Densitometry measurements made on the image are directly related to concentration of radioactivity and hence, by conversion, to the concentration of drug-related compound.
By the 2000s, as phosphor IP scanning was refined and perfected, QWBA became the widely accepted technique to conduct tissue distribution studies submitted to the FDA for radiation dosimetry calculations. See image in PDF.
SIDEBAR—HISTORY OF QWBA
WBA (whole body autoradiography) replaced LSC and other techniques that analyzed homogenized tissues and was the first technique that could precisely identify the location of drug entry and activity.
1954—Whole body autoradiography pioneered by Sven Ullberg, who applies labeled 35S-penicillin to mice and exposes whole body sections to X-ray film.
1975—Phosphor imaging technology introduced, making WBA quantifiable (QWBA); analysis of the image plates is known as radioluminography.
1995—Refined techniques yield digital images from whole body sections, allowing QWBA to be used in drug discovery/distribution studies. The Society for WholeBody Autoradiography forms to promote use over organhomogenate methods.
1997—QWBA is approved by the Japan Health Ministry for preclinical ADME study data.
2020—Most regulatory agencies and drug developers recommend ADME data be based on QWBA methods.
Figure 2. A library of images produced by QWBA techniques. Note the image detail and areas of radioactivity indicated by the arrows. Densitometry measurements made on the image are directly related to concentration of radioactivity. See image in PDF.
Advantages and Limitations of QWBA
The greatest advantage of QWBA over tissue counting methods (i.e., LSC) is direct quantitative measurement. Organs are not removed, which means the behavior of a drug can be studied in situ. This provides the true picture of a drug’s spatial distribution, making it possible to visualize where a drug travels and how long it takes to get there.
The advantage of QWBA (where the A stands for “autoradioluminology”) over traditional WBA (where the A stands for “autoradiography”) is the much wider dynamic linearity, which makes quantitation possible. Autoradiography produces higher-resolution images, but the exposure time to obtain those images is much longer (generally weeks). By contrast, autoradioluminography produces lower-resolution images, but exposure times are much shorter (days and even hours) because the sensitivity of the sensor is several hundred times higher than X-ray films.
The specificity of the autoradioluminographic method is that it uses image files instead of the actual sample. The grayscale digital images created in the process are calibrated using image analysis software so that different grayscale levels represent a quantitative value of radioactivity, typically reported as nanocuries (nCi) or microcuries (μCi) of radioactivity per gram of the tissue. These values can then be converted into drug equivalents per gram of tissue. These digital images can also be stacked together to create a 3-D model of the distribution in the animal models.
Methods of quantification are based on densitometry analysis, which requires that radioactive calibration standards (typically blood) with known concentration are exposed alongside the whole-body sections. A standard curve is generated, making it possible to quantify the radioactivity concentration in specific organs and other anatomical structures Another advantage of QWBA is the reusability of the
phosphor imaging plates. After an imaging session is completed and all data has been recorded, the plates are exposed to visible light, which resets the crystals and leaves the plate ready for another exposure. Because the background level on the next exposure is essentially zero, the image plate can more accurately detect lower levels of radioactivity than X-ray film.
Despite these compelling benefits, QWBA does have some drawbacks. One of the biggest is the inability of the technique to distinguish the parent drug from one of its many metabolites or even degradation products. This issue is compounded by the dehydration process used to prepare the samples, which can cause volatile metabolites to be lost before imaging can occur.
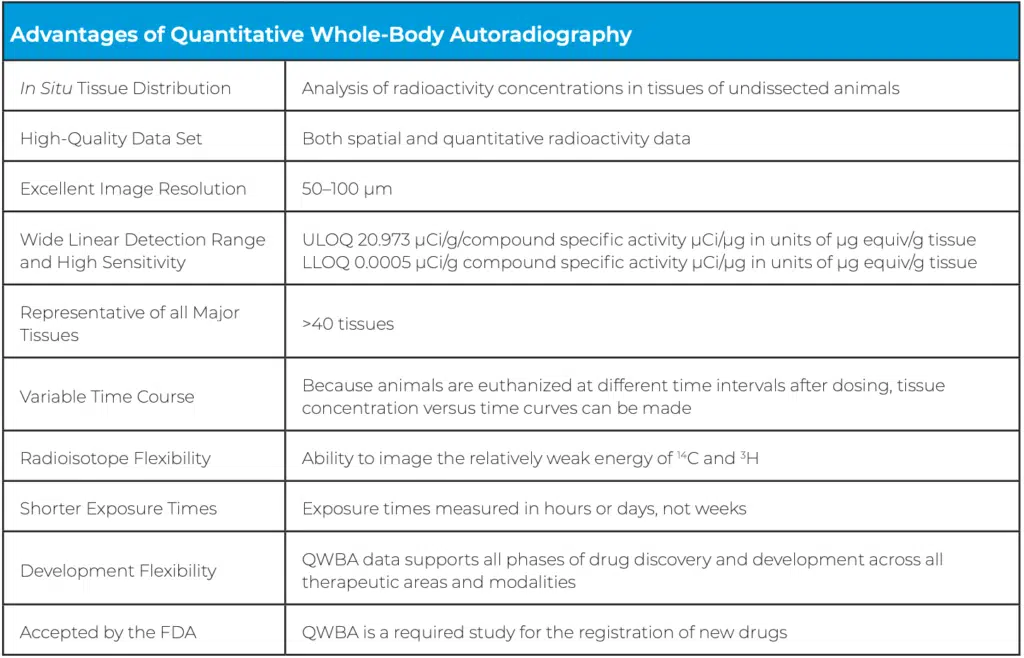
Another limitation is that QWBA is challenged to evaluate short-lived isotopes. For these types of isotopes, such as 90Y, 11C or 18F, which have very short half-lives, exposure may need to take place before dehydration occurs, with the specimens still frozen. This introduces technical challenges that can complicate the procedure. The setup costs to perform QWBA are substantial because of the advanced instrumentation required. For example, cryomacrotomes (Figure 3) can be significant capital expenses, as can phosphor imaging scanners. Plus, a team of specialists, trained in the processes and procedures of QWBA, are needed to perform sample preparation and analysis.
Figure 3. A typical cryomacrotome, used for sectioning animals in QWBA
Finally, it should be noted that the freezing process inherent to QWBA also nullifies it as a quantitative tool at the microscopic level. That’s because the freezing cycle in QWBA occurs too slowly, which allows ice crystals to form, damaging cellular structures. For cellular distribution studies, then, other techniques are required, though QWBA can be used in coordination with other bioanalytical techniques to provide a complete picture for ADME studies. Two closely related histological techniques — microautoradiography (MARG) and quantitative autoradioluminography (QARL) — are covered later in this paper.
QWBA Providers: What to Look for in a CRO
Given that QWBA is the gold standard for ADME studies conducted by the pharmaceutical industry, any major contract research organization will list this specialized service as part of its offering. A quick scan of several CRO websites reveals that QWBA services are typically described under headings such as Laboratory Sciences > DMPK/ADME; Lead Optimization > In Vivo ADME; Drug Candidate Evaluation > DMPK > hAME Study Services; and Contract Services > Radiolabeling & In Vivo ADME/PK Studies.
In addition to the variation in how the content is organized and labeled, CROs also describe their QWBA services differently, with most discussing their QWBA offering in vague terms. They often tout their experience, mention their expertise with specific isotopes and make claims about cost-effective and efficient ADME studies. What’s missing is a detailed presentation of what differentiates one provider from another. Do they prepare samples in a specialized way that yields quantitative and accurate results? Can they maximize the resolution achieved by the system and produce clear images that enhance data collection? Can they demonstrate their ability to generate reproducible and precise results? Do they have a proven track record with regulatory agencies?
In all fairness, due to the proprietary nature of this information, the answers to most of these questions are unlikely to be published on a public website, but drug discovery and development teams will want to ask these questions as they evaluate various providers and certainly before they sign any contract with a CRO for a specific tissue distribution study.
Here are some of the most important details to understand about a CRO that offers QWBA services:
FREEZE PROTOCOL
Because freezing biological samples can lead to the destruction of tissues and cells, strict freezing protocols for QWBA should be followed. Most labs freeze animals in hexane and solid CO2, though some also use methanol or heptane and solid CO2 or liquid nitrogen. More important is the freeze time, which should optimally fall between 15 and 30 minutes in a hexane/solid CO2 mixture. Shorter freeze times and higher freeze temperatures may allow expansion of and cracking of the specimen or an area of centralized translocation of water during the freeze process, translocating the radioactivity. Longer freeze times can reduce the resolution of autoradiography due to cell lysis or leaching of radioactivity from tissue compartments.
Another important consideration is body positioning during freezing. A positioning frame is a convenient way to hold specimens in the correct posture, but it’s also possible to align an animal dorsally using clamps and forceps. Either way, to get the best data, the specimen should be straight during freezing to ensure reproducible sectioning.
CALIBRATION STANDARDS
The traceability of calibration standards is important because it’s the calibration process that makes quantitation possible in QWBA. When data from a scanned cryosection is compared against a calibration curve, the precise concentration of radioactivity — and therefore concentration of drug — can be determined. Even if a CRO doesn’t publish its standards, it should be able to provide them upon request.
There are two ways for a lab to set up calibration standards — it can prepare its own standards using blood, plasma, liver or other tissues as the matrix or purchase commercial standards. CROs that prepare their own standards offer some advantages. The biggest is that they can provide more standards on their calibration curves, which allows more refined comparison data and higher and lower limits. At least five calibration standards should be used when constructing calibration curves for bioanalytical instruments, but a higher number of standards is better. Some labs offer 10 or higher, which is a valuable refinement in precision at the lower end of the curve.
CROs that purchase commercially prepared calibration standards should verify the purchased standards against blood or another matrix spiked with known concentrations of radioactivity.
QUALITY CONTROL
In QWBA, the thickness of cryosections can have a dramatic impact on the scanning results. A simple method to assure uniform section thickness is using quality control standards, which are sectioned and imaged along with the whole-body sections and are used to eliminate regions of differing thickness. A reliable CRO should be able to demonstrate that 97 percent or more of its sections meet its quality control standards, which proves reproducibility and makes comparisons within a study — and across previous studies — possible.
DOSE SITES AND ROUTES
CROs should also be able to provide dose-site data to complement tissue distribution data. For example, if drug efficacy or distribution is less robust than expected, there could be an issue with the drug delivery vehicle. If it’s not adsorbing efficiently, higher levels of the drug will be seen near the dose site. QWBA studies can be set up from the beginning to study these specific details, but good collaboration between sponsor and CRO is necessary, as is the CRO’s flexibility to handle such add-on requests.
The dose route is another key consideration. A high radioactive dose of an orally dosed compound with low bioavailability will improve tissue imaging. However, a high dose in the gastrointestinal tract could cause a higher number of artifacts. At the same time, a lower amount of radioactivity may be given for drugs administered intravenously because the test article will bypass absorption effects and be distributed more readily.
A good CRO will work with you to discuss these issues and develop a QWBA study that provides the high quality data you need to advance your drug development program.
EXPERIENCE WITH ANIMAL MODELS
Experience with a wide array of animal models is essential for any provider of QWBA services. This is especially relevant because variations among animals — such as pigmentation, gender, age, pregnancy, disease states — can impact tissue distribution studies. Experience with rodents, including Sprague Dawley, Fisher 344, and Wistar albino rats, as well as pigmented Long-Evans rats, is a given. But experience with a wider variety of rats, including Zucker Diabetic Fatty, Wistar Han, pups, and aged rats, is a plus. Likewise, commonly used mice strains, including CD-1, BalbC and C57BL/6, are standard mice in today’s models. Experience with transgenic mice and tumor-bearing mice is a plus. Then there are the animal species that may be more appropriate models of human PK and thus should be considered in addition to rodents. These include guinea pigs, cats, dogs, monkeys, pigs, and even non mammalian animals, such as reptiles, amphibians, birds, fish, and insects.
Another important question is whether a CRO can conduct lacteal transfer and placental transfer studies. Typically conducted on pregnant and nonpregnant rats and mice, this specialized type of QWBA study can provide information on placental and milk transfer and fetal exposure to new drugs.
A good CRO will bring all of these types of experience together and recognize variations of tissue distribution for your study, to give the highest precision tissue distribution data possible.
RECOGNIZABLE, CLEAR IMAGES
As previously discussed, the traditional imaging technique for whole-body autoradiography was X-ray film, which yielded high-resolution images. While these images provided excellent qualitative data on the distribution of a drug, they could not provide quantitative data. Plus, the X-ray images required cumbersome storage and retrieval systems.
Today, the most common QWBA imaging technique is phosphor imaging. In addition to providing tissue concentrations for human dosimetry prediction, the highresolution images yielded through phosphor imaging can be enlarged to provide more depth and detail of the distribution in key tissues and organs. Image quality, for these reasons, is critical, and a CRO should be able to provide a library of images demonstrating its ability to produce recognizable, clear images.
REGULATORY TRACK RECORD
QWBA is an integral component of the ADME package required for a New Drug Application (NDA) submission, so a CRO’s track record of accepted concentration data by regulatory agencies is a useful metric. Look for a lab that has proven experience with hands-on DMPK assay development and technical problem-solving and can describe successful collaborations with major pharmaceutical customers conducting in vitro/in vivo ADME studies, including radiolabeled mass balance, biliary excretion, PK, and QWBA studies. This experience should encompass preparation of preclinical DMPK reports according to regulatory standards and highquality regulatory documents including INDs, CTAs and NDAs.
QWBA and Microanalysis Techniques
The benefits of QWBA can be amplified when the whole-body imaging is combined with techniques that provide distribution data at the histological level. Two techniques commonly used in conjunction with QWBA are microautoradiography (MARG) and quantitative autoradioluminography (QARL). MARG focuses primarily on cell-level activity; QARL focuses primarily on organlevel activity. Used together, they can provide a powerful one-two analytical punch to supplement QWBA data.
Similar to QWBA, the MARG procedure begins with dosing an animal model with a radiolabeled substance, typically 3H, 14C, 35S, or 125I. After the animal is euthanized, however, the whole body is not frozen. Instead, technicians drain all the blood, remove organs and tissues, and then rapidly freeze them in liquid nitrogen. Then they cryosection the organs and tissues at the optimal cutting temperature for that particular organ or tissue (typically around -20°C) to obtain sections between 4 and 10 μm thick. Next, working in a darkroom, they mount the sections onto glass microscope slides precoated with nuclear photographic emulsion. Finally, they place the slides in a lightproof box at 4°C for exposure, which can take between one and 100 days depending on the radioconcentration.
After exposure, the slides are “developed” like film and then stained using conventional histological staining protocols. When investigators view the slides under a microscope, they can see radioactivity as dark areas (grains of silver) in the tissue. Similar to QWBA, this provides qualitative evidence of a drug’s presence and accumulation in organs and tissues.
What’s notable about MARG is the high resolution and sensitivity of the process. This results in detailed images that clearly show spatial localization of radiolabeled drugs at a tissue and cellular level. MARG is especially good at revealing the process of in vivo receptor binding in various cell types, which makes it useful at predicting specific drug targeting and elucidating cellular mechanisms.
With all of this said, it should be noted that special precision must be taken during MARG procedures. Slight variations in freezing, sectioning or exposure can lead to the development of artifacts and, consequently, false positive and/or false negative results. One of the most common artifacts, translocation, occurs when soluble radiolabeled substances move from one tissue location to another. Another drawback to MARG is the inability of the technique to provide quantitative data. To date, neither reliable calibration and quality control standards nor reliable upper and lower limits of quantitation have been established for MARG samples. Some researchers have attempted to develop semiquantitative MARG techniques based on counting the number of exposed silver grains using image analysis, but the reliability of this technique has not been proven.
When evaluating CROs that offer MARG services, it’s important to identify some key capabilities. In addition to experience with an array of soft tissues, a MARG provider should have strict freezing protocols in place to prevent ice crystal formation and the resulting artifacts that reduce resolution and confound results. Because tissue section collection under darkroom conditions is especially challenging, having specific darkroom techniques, which can be demonstrated or explained, is important. A lab should also have the proper equipment — and expertise — to obtain extra-thin sections that produce highresolution images and increase the accuracy of tissue location. Finally, a lab should have specialized techniques and skills, such as experience with thaw-mounting, to limit translocation of radioactivity. In thaw-mounting, the frozen section is carefully flattened with a paintbrush, then quickly picked up on a room-temperature glass slide, and the tissue is heat-fixed — a technique that requires some finesse.
It’s also advantageous to look for a CRO that can combine its MARG offering with QARL. Like QWBA, QARL uses phosphor image plate scanning, so it can provide quantitative data. Unlike QWBA, however, QARL focuses on single organs, not whole animal bodies, and it usually looks at one time point instead of multiple time points. After the organ of interest is frozen, in isopentane/liquid nitrogen to prevent ice crystal formation, it is sectioned to study the organ at three to four levels (e.g., kidney capsule, cortex, outer medulla, and inner medulla). Similar to MARG, the sections are thaw-mounted and heat-fixed on glass slides. Next, slides of sections are exposed to image plates (IPs). When the plates are scanned, typically at 50μm, high-resolution images of the organ are produced that can reveal both location and density of radioactivity. Figure 4 presents examples of images produced using MARG and QARL techniques.
One interesting add-on that can be attempted with MARG and QARL is book-matched or “sister” sectioning. In this technique, two sections are taken one right after the other. The first section is imaged using MARG techniques; the second is imaged using QARL. This allows investigators to compare data between the two images to get a robust qualitative/quantitative view of drug distribution in that tissue, at that moment.
The limitations of MARG have prevented it from becoming more widely adopted in drug discovery and development, but when it’s performed correctly and when it’s combined with other techniques, such as QARL, the results it provides can be extremely valuable in answering pivotal questions about a drug’s activity for pharmaceutical investigators.
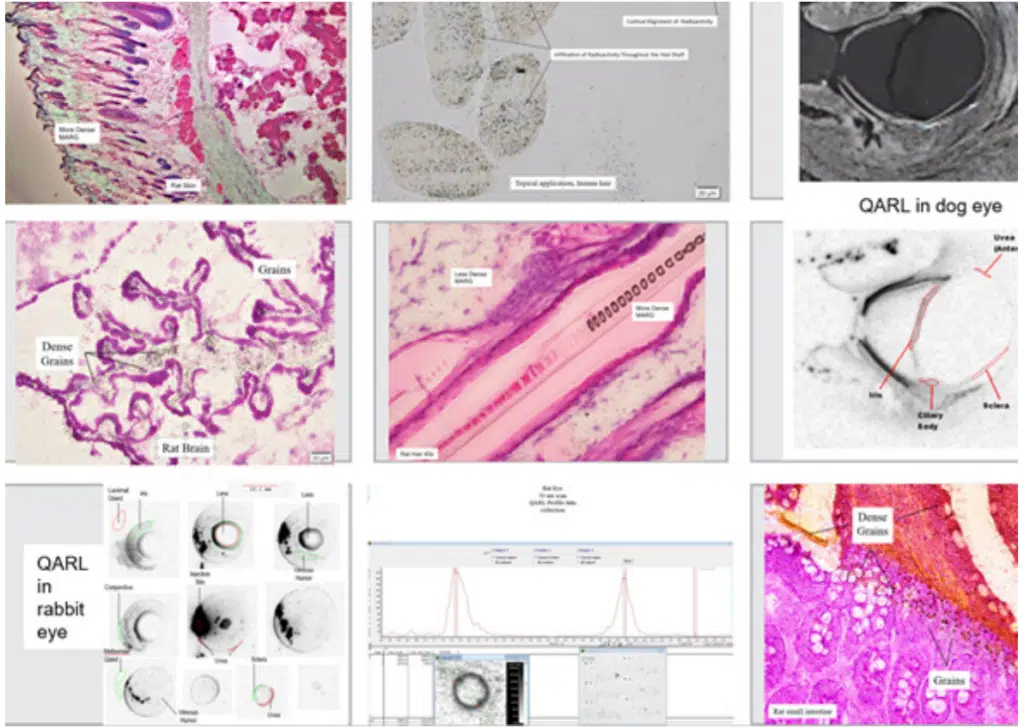
Figure 4. A library of images produced by MARG and QARL techniques. MARG images appear in color; QARL images appear in black and white. MARG can provide valuable qualitative data and, when used in conjunction with QARL, can quantify radioactivity at the organ and tissue level.
The Future of QWBA
As this paper has discussed, there are a number of methods available to pharmaceutical researchers looking to conduct ADME studies. Quantitative wholebody autoradiography (QWBA) and imaging mass spectrometry (IMS), including matrix-assisted laser desorption/ionization imaging mass spectrometry (MALDI-IMS), are two of the most common techniques that enable researchers to visually and quantitatively examine drug distribution in living systems. Some industry experts have argued that IMS should replace QWBA as the technique of choice when examining the behavior and activity of new drug and biotherapeutic entities.
At this time, however, regulatory agencies currently mandate the use of radiolabeled drugs to identify and profile human drug metabolism. To demonstrate that these radiolabeled test compounds can be administered safely to humans, investigators must show that human subjects will not be exposed to unsafe levels of radioactivity. Regulatory authorities currently recommend the use of QWBA — an imaging technique whose basic principles have been used for decades and whose procedures have been rigorously validated by many independent labs across the pharmaceutical industry for dosimetry testing.
Before IMS can replace QWBA, IMS practitioners will need to demonstrate and validate their ability to quantitate and profile drug metabolites. As a result, QWBA will remain the technique of choice to conduct ADME studies in the foreseeable future, especially when it is combined with other techniques that expand the qualitative and quantitative data available to researchers. These techniques could include IMS analytical processes like MALDI, but they will also include quantitative autoradioluminography (QARL) and qualitative microautoradiography (MARG).
Using such a balanced portfolio of imaging techniques, investigators can feel confident that they’re not simply obtaining images, but that they’re obtaining just the right images that tell a comprehensive and reliable story of their drug’s movement throughout the body.
QPS IS COMMITTED TO WORKING WITH YOU
QPS has extensive experience in supporting Drug Development. We understand the complexities, particularly with respect to managing and conducting global clinical trials, proper bioanalysis, and monitoring the pharmacokinetics of drug candidates. We are committed to working with you personally to advance your product for the benefit of patients worldwide.
BROAD ACCESS
QPS provides clients with broad access to our preclinical and clinical development capabilities. Clients also benefit from our experience in preclinical and clinical development of a diverse portfolio of treatment modalities for a wide range of trails indications. Our preferred vendor agreements also provide for the establishment of clientdedicated units within our organization.
TIMELY DELIVERY
Partnering with QPS will position your company for success, enabling timely, personalized delivery of your drug candidate portfolio to the marketplace.
REFERENCES
Appelgren, L.-E., The Technique of Whole Body Autoradiography—Some Examples of Applications, Upsala Journal of Medical Sciences, 91:3, 233-238 (1986)
Leblans, P., Vandenbroucke, D. & Willems, P. Storage Phosphors for Medical Imaging. Materials, 4(6); 2011, 1034–1086. DOI: 10.3390/ma4061034
Marzorati, S., Messina, M. & Ghiglieri, A. Autoradioluminography, a powerful and reliable tool for drug development: Accelera’s experience. Journal of Labelled Compounds and Radiopharmaceuticals, 2019; 62:776–784.
Nakajima, E. Radioluminography, a New Method for Quantitative Autoradiography in Drug Metabolism Studies. Radioisotopes, 42 (1993), 228–236.
Okuyama, M., Hatori, Y. & Shigematsu, A. Autoradioluminography, a novel quantitative method of TLC-autoradiography. Biological and Pharmaceutical Bulletin, 17(5), May 1994, 559–563. DOI: 10.1248/bpb.17.559
LARSSON, B., Ullberg S., Whole-Body Autoradiography, Univeristy of Uppsala, Uppsala, Sweden Received for publication June 30, 1980 (OA 80-227SA) 0022- 1534/81/0202 161002.50 The Journal of Histochemistry and Cytochemistry Copyright © 1981 by The Histochemical Society, Inc. Vol. 29, No. IA, pp. 216-225, 1981 Printed in U.S.A.
QPS, LLC Quantative Whole-Body Autoradiography (QWBA) DMPK Guidance Document: https://www.qps.com/service/preclinical/quantitative-wholebody-autoradiography-qwba/
Solon, E. & Krausb, L. Quantitative whole-body autoradiography in the pharmaceutical industry: Survey results on study design, methods, and regulatory compliance. Journal of Pharmacological and Toxicological Methods, 46 (2002) 73–81.
Solon, E.G., Schweitzer, A., Stoeckli, M. & Prideaux, B. Autoradiography, MALDI-MS, and SIMS-MS Imaging in Pharmaceutical Discovery and Development.
The AAPS Journal, 12(1), March 2010 (# 2009), 11–26. DOI: 10.1208/s12248-009-9158-4
Solon, E.G. Whole-Body Autoradiography and Microautoradiography in Drug Discovery and Development. In A.V. Lyubimov (Ed.), Encyclopedia of Drug Metabolism and Interactions (pp. 1–34). John Wiley & Sons, Inc.
Stumpf, Walter E. Drug Localization in Tissue and Cells Receptor Microscopic Autoradiography A basis for Tissue and Cellular Pharmacokinetics, Drug Targeting, Delivery, and Prediction Copyright 2003
Library of Congress Control Number 2003105179 ISBN 0-9740515-0-0 IDDC – Press, International
Institute of Drug Distribution, Cytopharmacology and Cytotoxicology
Ullberg, S., Autoradiographical Distribution and Excretion Studies with S35-Labelled Penicillin Proceedings of the Society for Experimental Biology and Medicine, Volume: 85 issue: 4, page(s): 550-553 Issue published: April 1, 1954